As you read these words on your device's screen, billions of tiny, microscopic particles called electrons are scurrying through the intricate pathways of the device's circuitry. This precise dance of the electrons at the microscale makes it possible for you to switch the device on, connect to the internet, open this page and scroll through it--all while countless other tasks run seamlessly in the background of the device. Although we rarely think about electrons every time we turn devices on and off throughout the day, just over 120 years ago, physicists around the world were debating the very existence of these particles.
The Crookes tube
If you've ever wondered how neon signs produce their constant captivating glow, you've already come close to understanding how an early discharge tube called the Crookes tube works. This single experimental apparatus, used in various ways, led to several breakthroughs in the late 19th-century Physics, including the discovery of the electron.
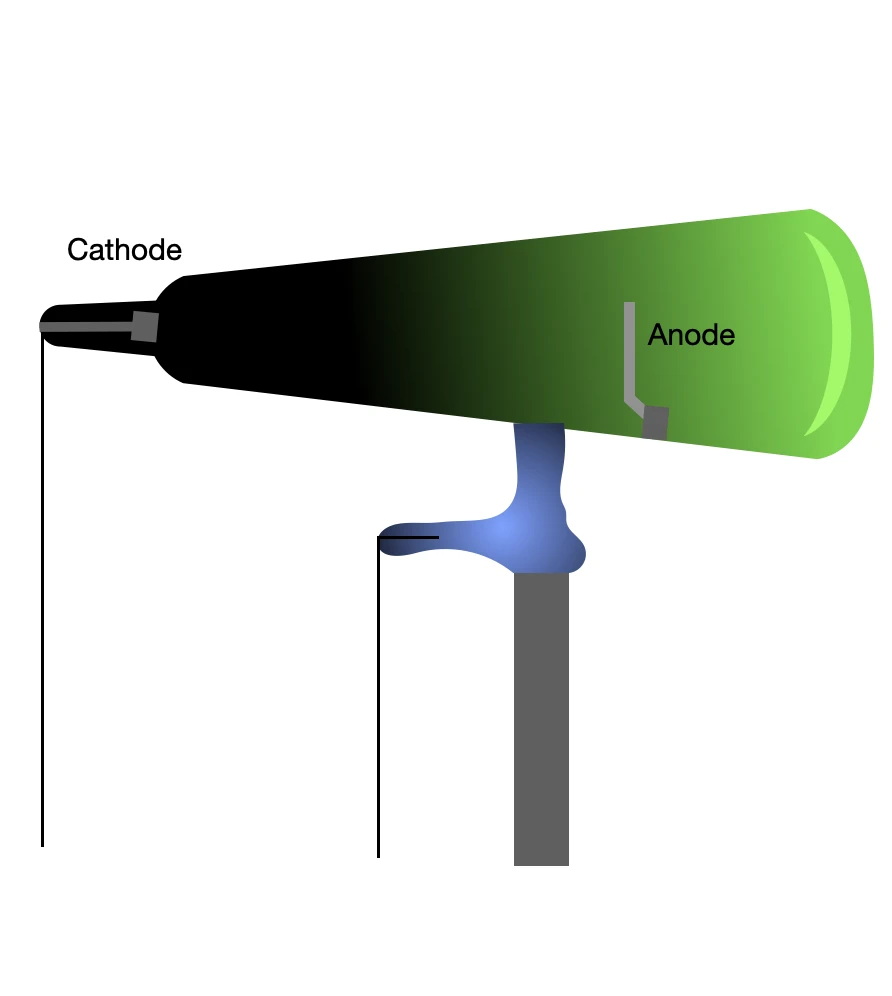
A Crookes tube consists of a glass bulb from which most of the air has been pumped out. The ends of the bulb are fitted with electrodes--metal terminals--between which a high voltage is applied with help of a battery. If the air pressure inside the tube remains high, meaning only a small portion of the air has been removed, the gas inside the bulb begins to glow under the high voltage--essentially the same principle behind the neon signs.
Historically, the earliest experiments with the Crookes tube involved poorer vacuums, but over time, the vacuum quality improved. While it might seem that the emptier the tube gets, the less interesting the observations would become--after all, who gets excited by observing a vacuum?--the reduced air pressure actually revealed new phenomena. One such discovery was cathode rays.
The cathode rays
As the vacuum in the tube improved, the gas glow became fainter and eventually disappeared, even though a high voltage was still applied across the electrodes. This was expected. However, a new greenish glow appeared on the glass just behind the anode (the positive electrode) and it persisted as long as the voltage was maintained.
It seemed that an invisible beam was emitted at the negative electrode, the cathode, that was travelling towards the anode, striking the glass behind it, causing the glow. These mysterious beams became known as cathode rays.
Throughout much of the late 19th century, physicists were eager to understand the true nature of cathode rays. Some believed them to be a form of light, while others thought they are beams of matter particles. There was no consensus among physicists about which of the two very different explanations was correct. Supporters of the light theory were at a disadvantage, as they had never seen light behave in such a way. JJ Thomson, an English physicist, belonged to the group that believed cathode rays were streams of material particles.
Cathode rays carry negative charge
In the early 1880s, Heinrich Hertz had attempted to determine whether the cathode rays were electrically charged by passing them through an electric field, but he observed no deviation in the beam. Based on this, he concluded that the rays are electrically neutral.
An electric field is a region of space around charged objects where other charged objects experience attraction or repulsion--similar to how when magnets push or pull each another via magnetic fields. If cathode rays were electrically charged, they would respond to an applied electric field by deflecting one way or the other. If they were positively charged, they'd deflect towards the negative plate and if negatively charged, they'd deflect towards the positive plate.
Although Hertz observed no deflection of cathode rays due to electric fields, it was found that the rays could be deflected by magnetic fields. In simpler terms, if a bar magnet were brought near a beam of cathode rays, the rays would curve.
Jean Perrin, a French physicist, later attempted to verify Hertz's findings using a clever set up. He discovered that cathode rays do carry a negative charge. Perrin allowed the rays to strike a metallic cylinder placed inside the Crookes tube, which was connected to an electroscope. Every time cathode rays entered the cylinder, the electroscope detected a negative charge.
An electroscope typically consists of a pair of gold leaves that are free to move apart when charged. Through this set up, Perrin concluded that cathode rays were negatively charged. However, many physicists, particularly those who believed cathode rays were a form of light, were skeptical. They questioned whether it was truly the cathode rays that induced the charge on the electroscope, or if it could have been caused by some stray charge or ions created produced by the rays.
Enter J.J. Thomson
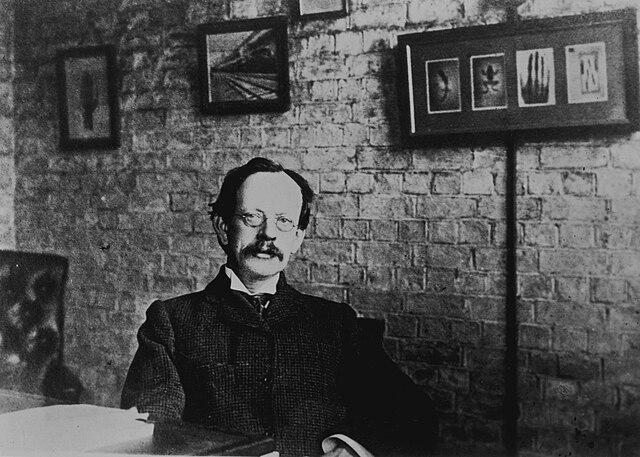
Thomson was determined to solve the mystery of the cathode rays, so he first set out to prove that they indeed carried a negative charge. He arranged slits to allow the cathode rays to pass in such a way that they could enter a cylinder connected to an electroscope only when a magnetic field bent the rays into the cylinder. He observed that a spike in the charge on the electroscope occurred only when the magnetic field bent the cathode rays sufficiently to let them enter the cylinder.
With this result, Thomson became certain that cathode rays carried a negative charge, further reinforcing his belief that they were composed of particles.
However, how was Hertz's conflicting observation to be explained--that cathode rays aren't deflected by an electric field? If cathode rays are indeed negatively charged, as Thomson had conclusively shown, they should have be deflected by an electric field. Thomson's experimental prowess lead him to realise that Hertz wasn't entirely wrong. The issue lay in the Crookes tube Hertz used, which didn't have a good enough vacuum. Under high voltage, the remaining air in the tube would begin to conduct electricity, effectively cancelling out the applied electric field. Thomson observed that the better the vacuum inside the tube, the lower the conductivity of the rarified air, increasing the likelihood of observing the cathode rays' deflection by the field.
Thomson's set up
To detect and measure the deflection of the cathode rays by an electric field, Thomson set up a pair of parallel aluminium plates separated by a small gap. A voltage could be applied across the plates by connecting them to a battery, creating an electric field between them.
The cathode rays were then directed through the gap between the plates, where the electric field is set up. The opposite end of the tube, where the cathode rays would hit, was coated with a phosphorescent material that glows when struck by high-energy cathode rays.
When the vacuum in the tube was sufficient, Thomson observed that the cathode rays were indeed deflected by the electric field. They deflected upwards when the upper plate was connected to the positive terminal of the battery and the lower plate to the negative. A downward deflection occurred when the voltage polarity was reversed.
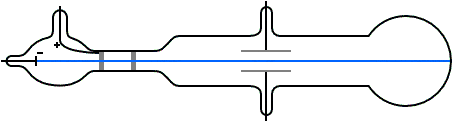
Thomson also noted that the reason a poor vacuum in the tube lead to no deflection, as Hertz had reported, was that under the high voltage, the residual air became ionised and started conducting. Positive ions accumulated on the negative plate, and negative ions on the positive plate, reducing and eventually cancelling the electric field between the plates. In the absence of the field, the cathode rays, though negatively charged, would surely travel undeflected.
To further confirm this conclusion, Thomson cranked up the voltage between the plates to a very high value and observed a significant bending of the cathode rays. However, within moments, a spark would jump between the plates, neutralising the electric field, causing the cathode rays to travel straight again. \
What are these particles?
Up to this point, Thomson's efforts had focussed on confirming whether cathode rays carried a negative charge. Once this was established, the next question arose: what exactly were these negatively charged particles? Could they be atoms, molecules, or something smaller?
To draw further conclusions it was necessary to determine some property of the cathode ray particles. Thomson chose to measure the ratio of the particle's mass to their charge. First, he applied an electric field to deflect the cathode rays, say upwards, and measured the angle of deflection.
Next, he turned off the electric field and introduced a uniform magnetic field inside the tube, generated by coils placed outside. The magnetic field also deflected the cathode rays, but in the opposite direction, downwards. He recorded this deflection angle as well.
Finally, Thomson applied both the electric and magnetic fields simultaneously, adjusting their strengths so that they produced equal deflections in opposite directions--one deflecting the rays upwards and the other downwards. As a result, the two effects cancelled each other out, and the rays travelled in a straight line, undeflected.
By calculating the balance between these opposing deflections, Thomson was able to determine the mass-to-charge ratio of the particles. He repeated the experiment for different field strengths, as well as with different gases in the discharge tube. He even used various metals for the cathode. Each time, he obtained the same value for the mass-to-charge ratio.
The conclusions
Thomson concluded that since the mass-to-charge ratio of the cathode ray particles was always the same, these particles must be present in all matter. In Thomson's own words "...this matter being the substance from which all the chemical elements are built up."
Further experiments revealed that the mass of these particles was about 2,000 times smaller than that of the lightest known atom at the time -- the hydrogen ion.
Thus, Thomson had systematically uncovered the existence of the first subatomic particle, which later came to be known as the electron (a combination of the words "electric" and "ion").
Today, we know that electrons are fundamental building blocks of all matter in the universe. If the universe were a giant Lego model, the electron would be one of its key Lego pieces. Electrons are the lightest particles known, with a mass of a billion-billion-billionth of a milligram. Moreover, electrons show no signs of an internal structure. If you imagined breaking matter into smaller and smaller pieces, you'd hit a dead end with the electron. In fact, electrons have no size--they are point particles with zero radius, meaning that no matter how much you zoom in on an electron, you'd always see it as a point, never as a ball or sphere of any size.
Every chemical reaction involves the rearrangement of electrons in atoms and molecules. All chemical properties of substances--from table salt dissolving in water, to iron rusting, car batteries providing power, the colours in fireworks, the function of LEDs, the burning of wood, and the flow of electricity through your house-- are fundamentally linked to the behaviour of electrons.
Today, electrons continue to play a crucial role in everything from the most basic chemical reactions to cutting-edge research in quantum computing. In a way, the journey that began with Thomson’s experiments with cathode rays continues to shape the future of science and technology, reminding us that the smallest particles can have the biggest impact.
The formula used by Thomson to compute the mass-to-charge ratio is \[\frac{m}{e}=\frac{B^2L^2}{2yE}\] where \(y\) is the vertical distance from the centre at which the electron beam hits the screen due to deflection by the fields \(E\) and \(B\). And \(L\) being the length of the region in which the fields are applied.